Diamonds Illuminate the Origins of Earth’s Deepest Oceans
Crystals could be the key to where our water came from, and what that means for finding life on other planets
/https://tf-cmsv2-smithsonianmag-media.s3.amazonaws.com/filer/75/39/7539348e-7d33-4671-bee5-79bc213b9931/juina_diamonds.jpg)
It was a spring day in 2009, and John McNeill had a pocket full of diamonds.
His PhD advisor, geochemist Graham Pearson, had sent McNeill to a lab in Vienna with a film canister that rattled with “ultradeep” diamonds. These weren’t the glittering gems of a jewelry store, but the rough, dull diamonds that had exploded towards the surface from a region hundreds of miles deep in the Earth’s mantle called the transition zone Miners in Brazil’s Juína district had discovered them several years before. Jewelers had passed on the cloudy stones, but for scientists, these precious minerals were windows into the deep Earth.
In a darkened laboratory, McNeill aimed a beam of light onto the surface of stone after stone, measuring the spectrum scattered by the diamonds and their impurities—hoping to find minerals in these inclusions that could tell him how these diamonds formed.
What he discovered instead gave scientists the first concrete evidence that there was water deep inside the Earth. If there was a vast reservoir of water molecules integrated into minerals hundreds of miles underground, it could explain how our blue planet evolved into one with plate tectonics and water, and eventually became habitable. Understanding that process isn’t just historical: The more we know about what made life possible on our planet, scientists argue, the more we’ll know about finding a habitable one outside of our solar system.
At the time, McNeill was a researcher at Durham University. When he and Lutz Nasdala, the scientist in whose lab he was working, compared the spectrum created by an impurity in one of the diamonds against a database of minerals, they found something surprising: A microscopic fleck of greenish crystal trapped within the diamond looked like it might be ringwoodite, a mineral that had only ever been synthesized in laboratories or found on meteorites. It had never shown up in material from Earth.
If it was, it would be a big deal. Synthetic ringwoodite was known to be able to incorporate water molecules into its structure. So this terrestrial sample might finally be able to settle a decades-long debate about the quantity of water trapped in the transition zone—a layer that stretches from 250 to 400 miles beneath the crust—and how it got there.
In the late 1980s, geophysicist Joseph Smyth from the University of Colorado, Boulder predicted that certain minerals in the mantle’s transition zone might have room in their structures for molecules of water. But because no one could drill that far down into the transition zone to take a direct look, most of the evidence for this was either theoretical or the result of laboratory experiments. Other scientists disagreed, noting that the way an earthquake's seismic waves moved beneath the surface—and the infrequency of deep earthquakes—predicted a dry transition zone.
McNeill’s diamond provided a pea-sized window into this hidden layer in the center of the Earth, allowing researchers to catch a glimpse of the composition of our planet.
About two years later, McNeill had graduated and Pearson had moved from Durham University to continue his research at the University of Alberta in Canada. On a winter day in 2011, in a windowless basement laboratory, Pearson’s colleague Sergei Matveev painstakingly suspended the ringwoodite-containing diamond inside an infrared microscope to analyze the contents of the tiny inclusion.
It took Matveev a few hours to position the diamond just right so he could take a measurement. But once he had it in place, it only took a few minutes to get their results: the ringwoodite contained water.
Matveev tried to stay calm, but Pearson was excited. He prefers not to repeat what he said the moment that he realized that theory and laboratory experiments could now be backed up by a direct observation of water from deep in the Earth’s mantle.
“It’s possibly not printable,” he says.
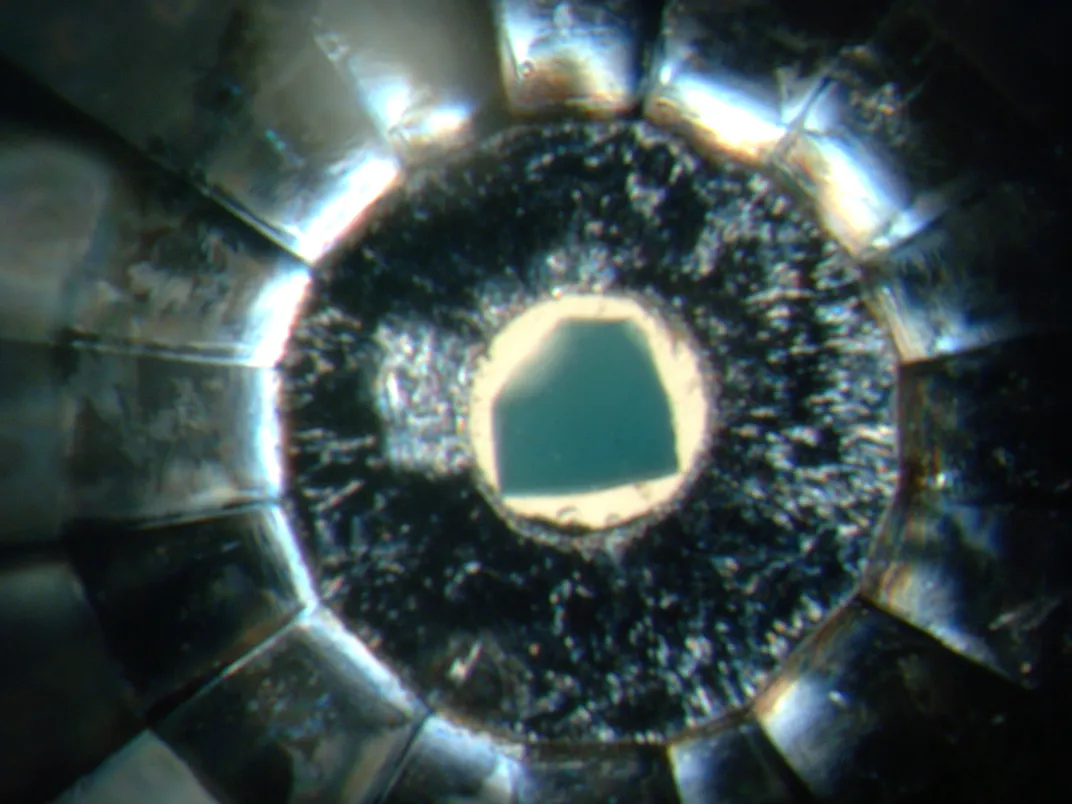
McNeill, Pearson and their colleagues published their discovery in the journal Nature in 2014, but the question remained: how representative was this tiny diamond of the entire transition zone? The two scientists were careful to note that their paper provided evidence of water only in the small pocket of the mantle where this diamond had formed.
If this tiny ringwoodite sample was truly representative, then the transition zone could contain as much water as all of Earth's oceans—possibly more. And if it did, it could help explain how plate tectonics move, forming mountains and volcanoes.
Geophysicist Steve Jacobsen from Northwestern University cautions against envisioning this water as Jules Verne’s subterranean oceans filled with sea monsters. Instead, he likens water in the transition zone to the milk in a cake. Liquid milk goes into the batter, but once the cake comes out of the oven, that liquid milk’s components are incorporated into the cake’s structure—it’s not wet anymore, but it’s still there.
And Jacobsen thought he had a way to find out just how much of this water was “baked” into the Earth beneath North America.
Inside our planet, incredibly hot and slightly viscous rock moves towards the surface in some places, while in others it oozes towards the core in a slow current called convection. As minerals like ringwoodite transit from higher to lower depths in the mantle, the high temperatures and pressures warp the mineral’s structure. Blue-tinged ringwoodite, for example, starts out as a green crystal called olivine near the surface, metamorphoses to ringwoodite in the transition zone, and changes into bridgmanite as it moves to the lower mantle. But unlike ringwoodite, bridgmanite doesn’t hold water.
Jacobsen theorized that if ringwoodite in the transition zone truly contained as much water as Pearson’s diamond suggested, then the water would ooze out of the ringwoodite as magma when the mineral was squeezed and heated to become bridgmanite.
So Jacobsen made ringwoodite that contained water in the laboratory, squeezed it between two diamonds in a pocket-sized vice called a diamond anvil press, and heated it with a high-powered laser. When he examined the results, he found that the high temperatures and pressures had indeed squeezed the water from the stone, creating tiny droplets of magma.
Jacobsen thought that if ringwoodite actually oozed water-rich magma as it was pressed into the lower mantle, then these patches of magma should slow an earthquake’s seismic waves—creating a kind of seismic signature for water.
So Jacobsen teamed up with seismologist Brandon Schmandt from the University of New Mexico to look for these signatures in the data collected by the National Science Foundation’s grid of mobile seismometers called the U.S. Array that was slowly moving east across North America. The researchers saw the seismic hiccups they predicted right where they thought they would—at the boundary between the transition zone and Earth’s lower mantle.
When he tries to describe what these results meant to him, Jacobsen is at a loss for words. “That was really the point where I felt that the last 20 years of my research were worthwhile,” he finally says. He and Schmandt had found evidence that water was trapped in the mantle’s transition zone underneath most of the United States, and they published their findings in the journal Science in 2014.
But there was still a big blind spot: no one knew where this water had come from.
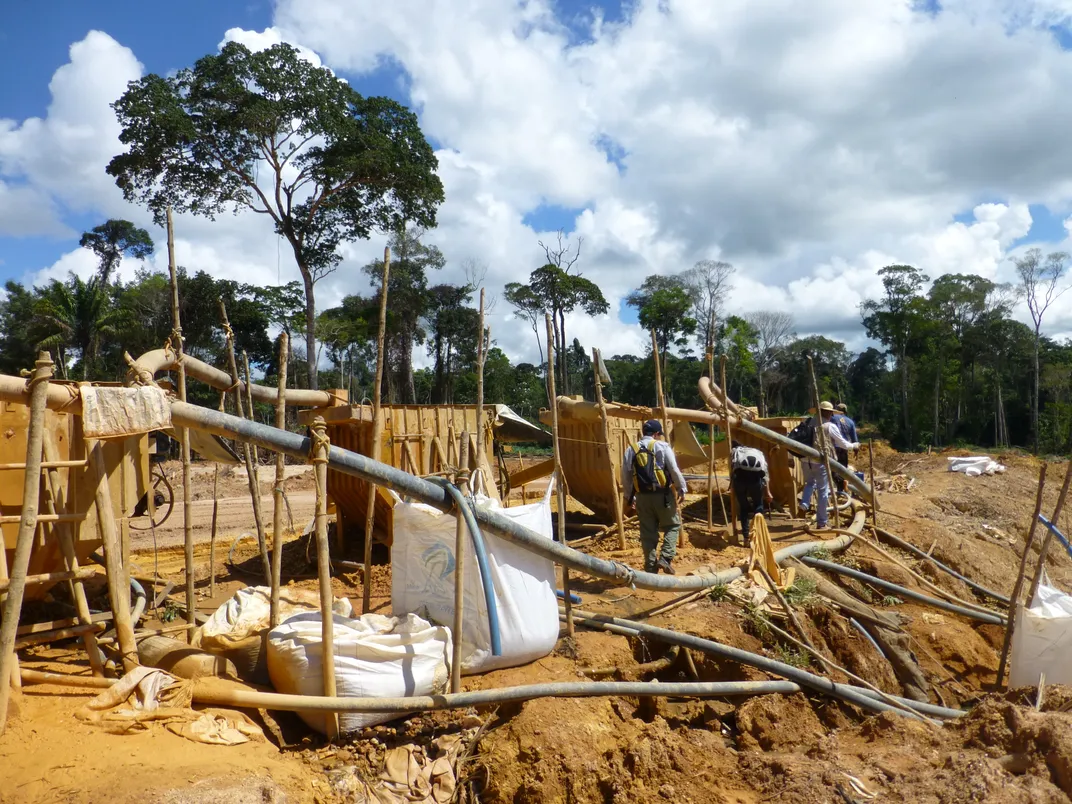
In September 2014, Alexander Sobolev set out to find “fresh” samples of rare, 2.7-billion-year-old lava rocks called komatiites, hoping to learn about how they formed.
Sobolev, a professor of geochemistry from Grenoble Alpes University in France, made his way through portions of Canada’s Abitibi greenstone belt with a hammer—tapping komatiites that looked promising, and listening carefully to the tinny percussion. The best ones, he says, make a clean and beautiful sound.
Sobolev and his colleagues Nicholas Arndt, also from Grenoble Alpes University, and Evgeny Asafov from Russia's Vernadsky Institute of Geochemistry collected fist-sized chunks of these rocks to take back to France. There, they crushed them and extracted the tiny green grains of olivine nestled inside before sending the olivine fragments to Russia to be heated to more than 2,400 degrees F and then rapidly cooled. They analyzed the molten and cooled inclusions trapped inside the olivine to understand what had happened to the plumes of magma as they shot up through the mantle.
Sobolev's team discovered that while these komatiites didn’t contain as much water as Pearson’s ringwoodite, it looked like the magma that formed them had picked up and incorporated a small amount of water as it traveled through the mantle—probably when it passed through the transition zone. This would mean that the mantle’s transition zone contained water 2.7 billion years ago.
This time point is important because there are a number of different—but potentially complementary—theories about when and how the Earth acquired its water, and how this water made its way deep into the mantle.
The first theory says that the young planet Earth was too hot to retain any water and that it arrived later, hitching a ride on soggy meteorites or comets. This water then slipped into the mantle when tectonic plates moved over one another in a process called subduction. The second theory says that water has been on our planet since the beginning—that is, ever since a cloud of gas and dust coalesced to form our solar system 4.6 billion years ago. This primordial water could have been trapped inside the Earth during its accretion, and somehow managed to withstand the young planet’s scorching heat.
So if water was in the Earth’s transition zone 2.7 billion years ago, Sobolev says, it means that either the movement of tectonic plates had to have started much earlier in the planet's history than scientists currently believe, or that water was here from the very beginning.
Lydia Hallis, for one, suspects that the water has been there all along. Hallis, a planetary scientist at the University of Glasgow, compared what she calls the different “flavors” of water in ancient rocks from the deep mantle and in regular seawater several years ago. While subduction mixes water into the upper levels of the mantle, the deepest portions stay relatively pristine.
Water is made up of two molecules of hydrogen and one molecule of oxygen. Sometimes, when it’s incorporated into rocks, it’s actually made up of one hydrogen and one oxygen, called a hydroxyl group. Different forms, or isotopes, of hydrogen have different molecular weights, and the heavier hydrogen isotope is known as deuterium.
Scientists think that at the spot in the nascent solar system where the Earth formed, water contained a lot more regular hydrogen than deuterium. But as water has persisted on the Earth’s surface, the lighter hydrogen molecules escaped into space more readily, concentrating deuterium in our atmosphere and oceans.
Hallis found that water trapped in stones from the Canadian Arctic that were formed by magma originating deep in the Earth’s mantle had a lower deuterium to hydrogen ratio than seawater. The ratio in those stones more closely resembled what scientists think primordial water looked like, suggesting that water was a component of the Earth’s mantle from the very beginning.
This doesn’t exclude the possibility that damp space rocks also smacked into the Earth and shared some of their water. But the debate rages on. “That’s how science works," Hallis says. "You’re right, until someone proves you wrong.”
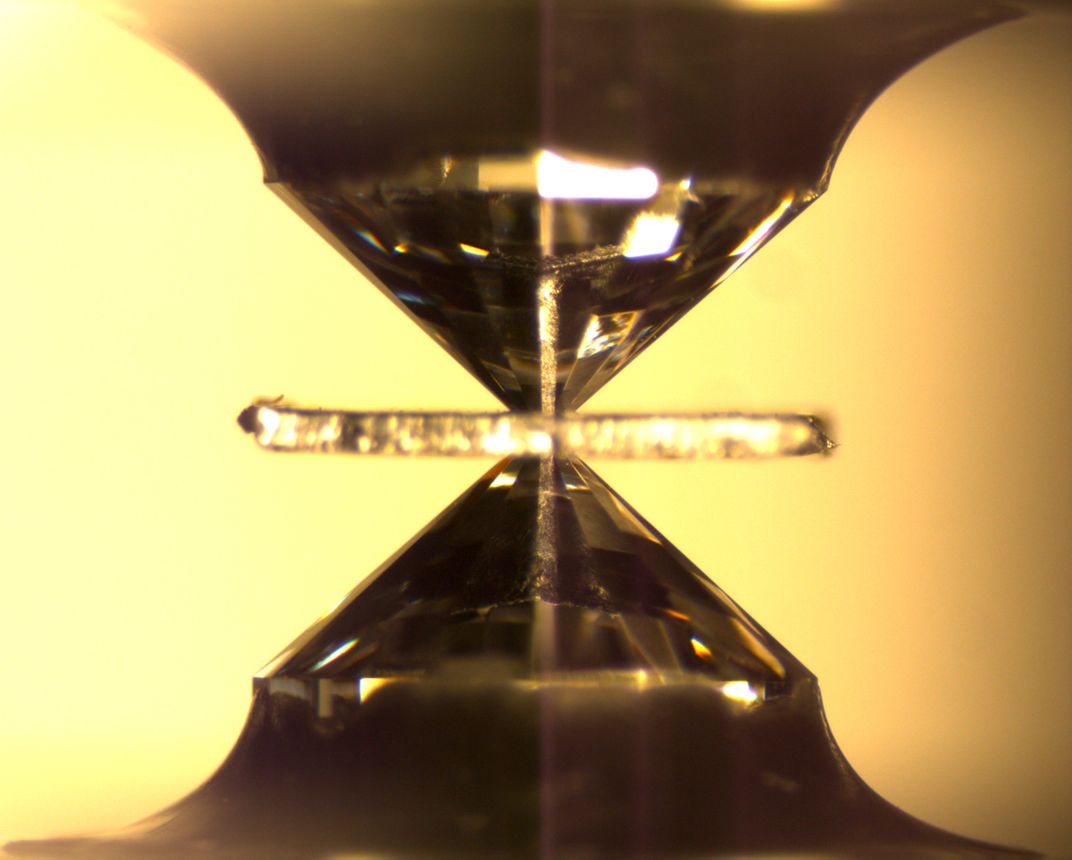
Pearson wondered if examining the ratios between deuterium and hydrogen in his ringwoodite inclusion might tell him more about whether the water in the transition zone was primordial, if it was there as a result of subduction, or whether it was a bit of both.
He recruited Mederic Palot—a geochemist currently at Jean Monnet University in France—to polish the diamond down to the ringwoodite inclusion so they could analyze the hydrogen molecules trapped inside. It was a risky process. Bringing a diamond up from such depths meant that its insides were under a lot of strain. Cutting and polishing the diamond could damage it and its inclusion beyond repair.
Palot was careful. He created a kind of heat sink made of dry ice so that the diamond wouldn’t overheat as he shaved tiny slivers off the mineral's surface with a laser. After each minute of polishing, he took the diamond over to a microscope to make sure that the precious ringwoodite inclusion was still there.
After 12 hours of polishing, Palot knew he was getting close to the inclusion. He checked the diamond under the microscope at 11 p.m.—almost there. He polished for another minute and then checked the diamond again. The inclusion was gone.
Palot frantically searched for it for an entire day, scouring the area around the microscope for a speck of ringwoodite smaller than a grain of dust.
He remembers the terrible feeling of having to call Pearson to deliver the news that the only sample of ringwoodite ever discovered that had been formed in the Earth was gone.
But Pearson was already thinking about the next project. “He said, ‘That’s game, we know that we gambled on that,’” Palot recalls. And then Pearson told him that they had another sample that might be interesting. He had recently made a trip to the same region of Brazil where the ringwoodite-containing diamond came from, and he brought back new gems—each with promising inclusions to study. Now, Palot, Pearson, Jacobsen and others are working together to analyze a diamond from even deeper within the mantle.
For Palot and each of these scientists, looking at crystals that emerge from deep within our planet is about more than identifying the ingredients that were baked into the Earth billions of years ago.
“This whole point is about life itself,” Palot says. “We know that life is closely related to water. If we know better the water cycle, we know better how life originated.”
And if we know how life originated on our planet, it could potentially help us find life—or life-sustaining conditions—on others.
Jacobsen adds, “We’re now discovering potentially habitable planets outside of our solar system. And the more we know about what a habitable planet looks like, the more we’ll be able to recognize them.”
Their search for water deep inside the Earth, Jacobsen says, has never been more relevant.
Learn about this research and more at the Deep Carbon Observatory.